How Old is the Grand Canyon?
A pile of rocks like those in the Grand Canyon does not reveal its age easily. But we have evidence of seas, mountain building, mountain erosion, more seas, more mountain building, more erosion, and more, and more, and more. The rocks involved are old friends—similar things are forming today. Using the principle of uniformitarianism—the present is the key to the past—we can make some estimate as to how long events take. The schists at the bottom were buried miles deep in mountain ranges and later brought to the surface by erosion, and even relatively fast erosion requires a million years to strip off a mile across a large landscape, for example.
The geologists of the 1700s, working primarily in Europe, pieced together stories such as this. They tried to estimate the times involved. One difficulty was that they could not tell how much time was in the erosional time gaps, or unconformities—was erosion fast, or slow? And they could not really unravel all of the stories in the oldest rocks because metamorphism had erased some of the stories.
These early geologists eventually estimated that the rocks told of events that required AT LEAST tens of millions of years to hundreds of millions of years. Just depositing the sedimentary rocks would take about that long, with much more time represented by the unconformities and the oldest really-messed-up rocks. This is deep time—the Earth is not just the historical thousands of years, or even the tens of thousands of years of ice layers and tree rings. History was written and trees grew on the relics of vastly greater histories. Looking back into that history, like looking over the cliff at the edge of the Grand Canyon, is one of the great joys of geologists. We live in a four-dimensional world, height, width, depth and history through deep time. We hope you are learning to enjoy some of this view over the cliff of time. In the next section, we will see just how high that cliff really is.
Radioactive Clocks
The techniques of layer counting and uniformitarianism are useful in dating, but the real workhorse these days is radiometric or radioactive dating. The Earth includes many different naturally occurring radioactive elements. An atom of a radioactive element eventually will spontaneously change to some other type of atom, by emitting radioactive energy, in ways that physicists describe and predict with incredible accuracy using quantum mechanics.
Radioactive decay occurs in various ways. The easiest to understand is when a nucleus splits into two parts, kicking out a part of itself. Remember that heat causes molecules in water to bounce around and occasionally evaporate; atoms or molecules in rocks are also bouncing around, but are so tightly bound that very, very few break free at the Earth’s surface. In a vaguely analogous way, the protons and neutrons in the nucleus of an atom are always wiggling and bouncing around; most nuclei are so tightly bound that this wiggling doesn’t change anything, but some types of nuclei are weakly enough bound that occasionally some protons and neutrons “evaporate.” We call those types of atoms that “evaporate” radioactive, and those that do not stable. (A real nuclear physicist would probably yell at us because we oversimplified too much, especially because radioactive decay properly is a quantum-mechanical process and not really like heat, but we hope this will do for introductory geology. We can guarantee that there are physics professors who would love to teach you about the real physics of this.)
Commonly, a nucleus that “evaporates” emits a group of two protons and two neutrons, which is the nucleus of a helium atom and also is called an alpha particle, for historical reasons. Other types of radioactive changes also occur, including the splitting of a nucleus into nearly equal-sized chunks, the change of a neutron to a proton plus an electron that is emitted, or the capture of an electron by a proton to change into a neutron. All of these change the type of atom from one element to another. All are explainable by well-known physical principles, and all are as natural and regular as the downward fall of your pencil if you drop it off your desk.
The behavior of any one atom is not predictable, but the average behavior of large groups is easily predictable with great accuracy. Suppose you start with a sample containing some atoms of a radioactive type, and you watch for some specified time such as one hour, or one year. The basic rule of radioactive decay is that you will see more radioactive atoms decay if you started with more radioactive atoms. (Really, it is that simple. We give you the math in the Enrichment, in case you want to prove it to yourself.) If you start your stopwatch when you have some number of a given type of radioactive atom, and stop the watch when half have changed, you will have estimated the half-life of the radioactive type. Each radioactive isotope has a distinctive half-life, which can be measured in the laboratory. (Note that you do NOT need to wait for an entire half-life to measure it. As shown mathematically in the Enrichment section, you need to wait only long enough for enough atoms to change to be measured accurately, a useful result when dealing with types that have long half-lives.)
Suppose you start with 2000 atoms of the parent type. These decay into offspring (most textbooks refer to these offspring as daughters). After one half-life, 1000 parent atoms remain and 1000 offspring have been produced. After another half-life, half of those 1000 parent atoms have changed to offspring, leaving 500 parents and giving 1000+500=1500 offspring. After a third half-life, half of the remaining parents have changed, so that now only 250 parents remain and 1500+250=1750 offspring have been produced. During the fourth half-life, half of the remaining parents decay, leaving only 125 parents and giving 1750+125=1875 offspring.
Now, we really need to deal with large numbers, so add ten zeros to the end of each of the numbers in the previous paragraph. Such numbers of radioactive atoms are common in even relatively small samples of rock; the total number of atoms in a fist-sized chunk of rock is about 1 followed by 24 zeros.
As noted, there are many different parent types with different half-lives. Some half-lives are very short—seconds or less. Others are very long—billions of years or more. Some of the radioactive parents are left over from the explosions of stars that produced the stuff of which the Earth is made. Other radioactive parents are created by cosmic rays that strike atoms on Earth. Some radioactive decays produce offspring that are themselves radioactive parents for a further generation, and several such decays may be required to produce a stable offspring. And radioactive decays may damage neighboring atoms, producing new radioactive types.
Telling Time
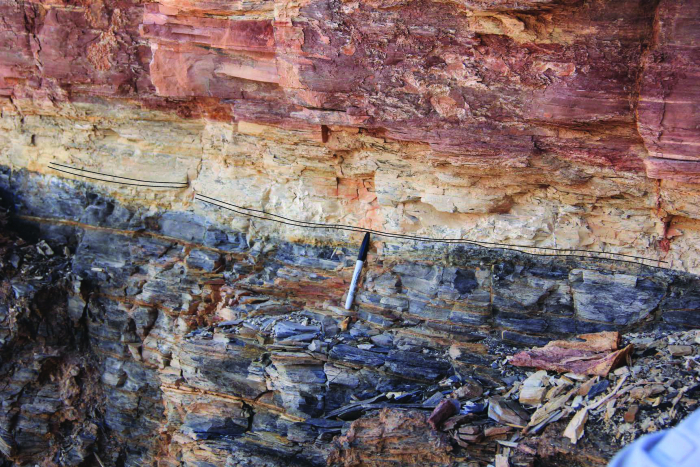
Consider the example of potassium-40 and argon-40. Argon-40 has 18 protons and 22 neutrons in its nucleus, for a total of 40 particles. Potassium-40 has 19 protons and 21 neutrons, also totaling 40. Potassium-40 is a parent with a half-life of 1.3 billion years. Potassium is abundant on Earth, and occurs in many common minerals, and some of the potassium is the radioactive parent potassium-40. The offspring, argon-40, is a gas. If lava flows out on the surface of the Earth, the argon escapes. Thus, a lava flow will start with some parent potassium-40 but no offspring argon-40. As time passes, the potassium-40 breaks down to argon-40, which builds up in the rock. If today the rock has as many potassium-40 as argon-40 atoms, then one half-life has passed since the lava cooled, and the rock is 1.3 billion years old. Whatever the ratio is, the math is not that difficult and gives the age.
It is possible for argon-40 to leak out of the mineral. If it does, we will think that the lava cooled more recently than it really did. But if leakage is occurring from a mineral grain, then the outside of the grain will contain less argon-40 than the inside does, and this can be measured, revealing the problem. A mineral grain that grew in slowly cooling melted rock far down in the Earth and that then was erupted may have begun trapping argon-40 before the eruption occurred, in which case the age obtained will be the time when the grain started growing rather than the time when the eruption occurred. Scientists do not blindly apply dating techniques; they think about what is being measured, and apply a little common sense.
Keeping Time
Clearly, we can test radioactive dating against written histories and annual layers, and we can test against the sort of uniformitarian calculations that the early geologists made on how long it would have taken to deposit the rocks we see today. Furthermore, we can test different radioactive isotopes against each other—a rock can be dated by potassium-argon, but also by others including uranium-lead and rubidium-strontium. All of these agree beautifully; the ages assigned to geologic events are based on multiple independent techniques that yield almost exactly the same age for those events.
In some of the stranger corners of the internet you may find people suggesting that maybe radioactive decay occurred at some different rate in the past, and even some of the freer-thinking physicists have suggested slight changes in physical “constants” over time, perhaps affecting radioactive dating. We can be confident, however, that no large changes have occurred that would significantly change the results discussed in this course. The agreement among written histories, annual-layer counts, uniformitarian calculations, and multiple independent radioactive techniques does not allow major changes. Furthermore, because radioactive decay depends on the forces controlling the stability of atomic nuclei, and those forces are involved in all sorts of other processes including energy generation in the sun and other stars, any major change in the radioactive decay in the past would mean that we would not be here today—the sun would have turned off or blown up already, something we know did not happen. (See the Enrichment if you want a little more on these topics.)
The Age of the Earth
The oldest rocks found on Earth are about 4 billion years old, and some of those contain mineral grains recycled from slightly older rocks. The active Earth has almost certainly erased the record of its very earliest rocks. Meteorites probably formed from the solar nebula at about the same time as the Earth did, and since then have fallen on the Earth. The oldest meteorites are about 4.6 billion years old, and that is our best estimate for the age of the Earth. Careful analyses of the changing lead isotopic ratios over time (from decay of uranium) also yield that number for age of the Earth. And 4.6 billion years is, indeed, deep time.
Vintage Video: Supergroup Part 1 (2:02 minutes)
This video takes you "live" to the Grand Canyon Rim (on a very windy day), where you will join Dr. Alley in a firsthand look at "deep time." (If that clip leaves you wanting more, "part 2" is also available as an optional enrichment). So, enjoy your visit to the Grand Canyon and your walk up through time. We hope you find Dr. Alley's play-by-play commentary and his incisive post-game analysis helpful in explaining what the Earth has been doing these past 4.6 billion years.
Optional Enrichment
No, this vintage video won't be on the quiz!